Introduction
Multiple Sclerosis (MS) is pathologically characterized by immune-mediated inflammation, demyelination, and axonal damage in the central nervous system (CNS) [1]. Based on the revised version of classification of the MS clinical courses, two main forms of the disease are considered: the relapsing-remitting MS (CIS: clinically isolated syndrome and RRMS: relapsing-remitting patients) and the progressive MS (PPMS: primary-progressive course patients and SPMS: secondary-progressive) [2]. In 85% of the patients, disease onset is characterized by a first acute clinical episode CIS, including optic neuritis, paresthesia, paresis, and fatigue [3], evolving into an RRMS course, and after a delay varying between 15 and 20 years, into an SPMS course, results in long-term disability. The remaining 15% of MS patients start with the PPMS [4, 5]. In MS disease, the ability of the immune system to set a balance between pro-inflammatory and anti-inflammatory responses is lost. Thus, it appears that an imbalance in Th1/Th2 and Th17/Treg cells and M1/M2 phenotypes of macrophages and microglial results in the progression of MS. In the following sections, we try to depict a comprehensive map of regulatory immune cells in inflammation. Then, we will discuss how the depicted map can address the challenges of biotherapeutics developments in MS disease.
Intervention of common lymphoid progenitor (CLP) cells' lineage in the immunopathogenesis of multiple sclerosis
Thelper (Th)1/Th2 differentiation imbalance and MS
Th1 cells produce neurotoxic interferon γ (IFN-γ) and tumor necrosis factor α (TNF-α) pro-inflammatory cytokines [6, 7]. Besides, brain-infiltrating Th1 cells can induce the differentiation and expansion of major histocompatibility complex II+ (MHCII+) microglia, probably by secretion of IFN-γ. MHCII+ microglia have considerable phagocytic and antigen presentation capacity than those of MHCII-microglia and are required to reactivate infiltrated Th cells [8]. The Th1 cells also produce high Fas ligand (FasL) levels that bind to Fas receptors, which are expressed on oligodendrocytes and neurons. Interaction of Fas-FasL induces apoptosis in cells leads to impaired myelin synthesis and neuronal damage [9]. Interleukin (IL)-12 and IFN-γ cooperatively trigger the expression of the master regulator of Th1 cells, T-bet, which in turn augments Th1 polarization by inducing additional IFN-γ and T-bet expression. Interference of IFN-γ in naïve T cell differentiation disturbs Th1/Th2 differentiation balance. Shifting the Th1/Th2 balance towards Th1 cells plays a key role in MS pathogenesis [10]. Conversely, Th2 cells produce a subset of anti-inflammatory cytokines, including IL-4, IL-5, IL-10, and IL-13, that could have beneficial effects on the clinical course of MS disease [11] (Figure 1).
Th17/Treg differentiation imbalance and MS
The Th17 cells are frequent in the initial phases of MS and experimental autoimmune encephalomyelitis (EAE). In addition, human Th17 cells produce CCR6 chemokine receptors supporting their increased migratory capacity in MS patients [12]. So, Th17 cells produce pro-inflammatory cytokines such as IL-17, IL-21, and IL-22 [13]. Moreover, the expansion of IL-17 producing Th17 cells has been correlated with the number of active plaques detected by magnetic resonance imaging. It implicates that the IL-17 production is tightly correlated with the MS progression [13-15]. Interaction of IL-17 with its corresponding receptors on various cell surfaces, such as T and B lymphocytes, mononuclear cell systems, and endothelial cells, triggers activation of nuclear factor kappa-light-chain-enhancer of activated B cells (NF-κB) [16-18] (Figure 2). Activated NF-κB stimulates pro-inflammatory cytokines such as IL-6, IL-8, TNF-α, and Granulocyte macrophage-colony stimulating factor (GM-CSF), chemokines like CCL20, CXCL1, and CXCL5 as matrix metalloproteinases (MMPs) [19, 20]. The Th17 cells also trigger apoptosis in neurons via Fas: FasL interaction [9, 13]. The transforming growth factor β (TGF-β) (at low concentration) in combination with IL-6, IL-1β, IL-23, or IL-21 induces the expression of RORγt, which is critical for the differentiation of Th17 [21, 22]. Macrophages and dendritic cells are the main sources of IL-6 and IL-23 cytokines [21, 23] (Figure 1). The impaired balance between the Th17 and Treg cells is correlated with the severity of MS symptoms [24]. The CD4+CD25+FoxP3+ regulatory T cells (Tregs), described as autoimmune response suppressors, finely tune the peripheral self-tolerance. They preserve homeostasis through the secretion of TGF-β and IL-10 [25]. In MS patients, expression levels of FoxP3 (mRNA and protein) are diminished while the suppressive function of Tregs is simultaneously impaired [26, 27]. Moreover, TGF-β reduces the production of inducible nitric oxide synthase (iNOS), which catalyzes nitric oxide production in macrophages and microglia [26, 28]. Moreover, High pathogenic Th1-like Th17 cells expressing both T-bet and RORγt are derived from Th17 cells in the presence of IL-12, TNF-α, and IL-1β. These cells produce lower amounts of IL-17A compared to classical Th17 cells but express high levels of IFN-γ. On the other hand, myelin-specific Th1-like Th17 cells have been identified in MS patients and EAE [29].
CD8+ T cells and MS
The CD8+ T cells, or cytotoxic T cells, are the most frequent T cell subsets in acute and chronic MS lesions [6, 26, 30]. The CD8+, not CD4+ T cells express high levels of P-selectin adhesion ligands, favoring their high capacity to be recruited at inflamed brain venules during the relapse phase in RRMS patients [31]. The CD8+ T lymphocytes recognize myelin peptides, presented by MHC Ι molecules, which express at high levels on neurons and oligodendrocytes in the CNS of the MS patients [6, 19]. The CD8+ T cells directly damage resident CNS cells, which have presented myelin-specific peptides by secreting cytolytic components (perforin and granzymes) [6, 19]. The CD8+ T cells also enhance CNS inflammation by the production of IFN-γ, TNF-α, and IL-17 cytokines [26, 32] (Figure 1 & 2).
In this regard, as demonstrated in figure 1, over-activation of Th1, Th17, and CD8+ T cells, is in favor of neuroinflammatory responses.
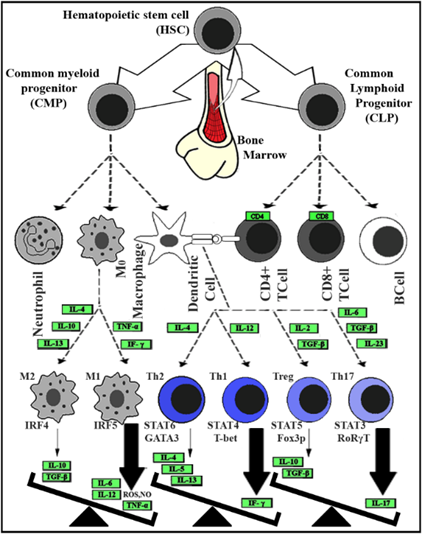
Fig. 1. The imbalance between inflammatory and anti-inflammatory immune cells and their responses
The involved cells are HSC: Hematopoietic stem cell, CMP: common myeloid progenitor, CLP: common lymphoid progenitor, CD4+ T cell: Activated T cell with CD4 on the surface, CD8 + T cell: Activated T cell with CD8 on the surface, DC: Dendritic cell, M0: non-activated Macrophage, M1/2: Activated Macrophage type I or II and (Th) T helper cell 1/2/17 and regulatory. The abbreviated protein names (represented by green rectangular) stand for; Cluster of differentiation (CD) 4 and 8, IF-γ: Interferon γ, TNF-α: Tumor necrosis factor-alpha, Interleukin (IL) types 2, 4, 5, 6, 10, 12, 13, 17 and 23, TGF-β: Transforming growth factor-beta, IRF4/5: Interferon regulatory factor4/5, STATs: Signal transducer and activator of transcription factors from either type of 3/4/5/6, GATA3: transcription factor that is encoded by the GATA3 gene, T-bet: A member of the T-box family of transcription factors, FoxP3: Forkhead box P3 or scurfin, RORγt: Retinoic acid receptor-related-orphan-receptor-C. The abbreviated molecular names stand for; ROS: Reactive oxygen species and NO: Nitric oxide.
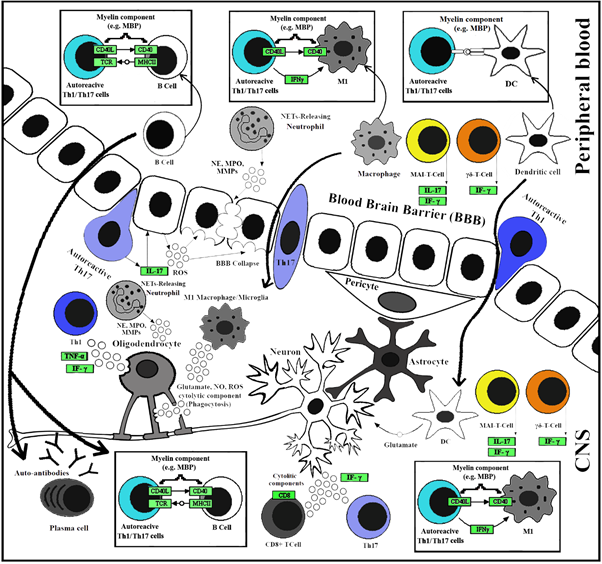
Fig. 2. Immunopathogenic mechanisms in MS
The involved cells are CD4 + T cell: Activated T cell with CD4 on the surface, CD8 + T cell: Activated T cell with CD8 on the surface, DC: Dendritic cell, M1: Activated Macrophage/Microglia type I, MAIT: Mucosal-associated invariant T, Th1: T helper cell 1, Th17: T helper cell 17 and γδ T cells. The abbreviated protein names (represented by green rectangular) stand for; Cluster of differentiation (CD) 4 and, 40, MHCII: Major histocompatibility complex II, TCR: T cell receptor, IF-γ: Interferon γ, TNF-α: Tumor necrosis factor-alpha, IL-17: Interleukin 17, MBP: Myelin basic protein, MMP: Matrixmetalloproteinas, MPO: Myeloperoxidase, NE: Neutrophil elastase. The abbreviated molecular names stand for; ROS: Reactive oxygen species and NO: Nitric oxide.
Natural killer T cells (NKT) and MS
Natural killer T cells share both T cells and NK cells properties that bridge innate and adaptive immunity. They express T cell receptors (TCR) and NK cell markers such as CD161 and CD94. NKT cells have been divided into two subpopulations, type I, invariant NKT (iNKT) cells, and type II, variant NKT (vNKT) cells, which their TCR recognizes glycolipids presented in complex with the CD1d, which is an MHC class I-like molecule expressed by antigen-presenting cells (APCs). NKT cells have various inflammatory and protective roles in MS owing to their cytokine production pattern. Activated NKT cells secrete significant amounts of cytokines such as IL-4, IL-10, IFN-γ, and IL-17. In a previous study, NKT cells produced IL-4 following attachment to α-galactosylceramide (α-GalCer)-CD1d complex, thus ameliorating EAE severity [33, 34].
γδ T cells and MS
The γδ T cells are a small subset of T lymphocytes in peripheral blood, which bridge the innate and adaptive immune systems and NKT cells. These cells express a distinct TCR heterodimer composed of γ and δ chains on their surface and respond to inflammation/infection by producing inflammatory cytokines [35]. Previous findings represent various roles for γδ T cells in EAE mice [36]. Moreover, alleviation of disease symptoms following depletion of γδ T cells in the EAE model, as well as, increment of IFN-γ producing γδ T cells in the relapse phase of RRMS patients, suggests that these cells play a critical role in the MS pathogenesis [37, 38].
Mucosal-associated invariant T Cells (MAIT) and MS
MAIT cells are a subset of innate-like lymphocytes and makeup to 10% of circulating T cells in adult humans. MAIT cells display a semi-invariant αβ TCR which recognizes microbial antigens presented on a non-polymorphic class Ib-related MHC molecule (MR1). Recently, it is reported that in MS patients, particularly during exacerbation of clinical manifestations, MAIT cells overexpress chemokine receptors such as CCR5, CCR6, CXCR6, and the integrin very late antigen-4 (VLA-4). In addition, the IL-17 and IFN-γ producing MAIT cells overpopulate in cerebrospinal fluid (CSF) of RRMS patients compared to their peripheral blood. These results point out the migration of MAIT cells into the CNS, with deleterious outcomes in MS patients [39, 40].
B cells and MS
B cells play important roles in the suppression and progression of MS development [41]. The involvement of B cells in MS pathogenesis was first identified by detecting oligoclonal bands (OCB) in the CSF of MS patients, which resulted from the elevated secretion of autoantibodies (immunoglobulin (Ig)G and IgM). OCBs (IgG and IgM) are detectable in 90% and 30-40% of MS patients, respectively, and are important biomarkers for MS progression. These autoantibodies bind to myelin fragments, which facilitates myelin and phagocyte interaction and results in complement-mediated demyelination.
B cells also act through antibody-independent mechanisms in the pathogenesis of MS. Likewise, B cells can act as APCs and activate effector T cells by presenting myelin antigens (e.g., myelin oligodendrocyte glycoprotein (MOG) and myelin basic protein (MBP)) to them. Many studies have reported an imbalance between pro- and anti-inflammatory cytokines (secreted by B cells) in MS patients. B cells secret high levels of IL-6, TNF-α, lymphotoxin alpha, and GM-CSF, as well as anti-inflammatory cytokines (e.g., IL-10, IL-35, and TGF-β) that comes up with pernicious and protective effects in different stages of the disease [42, 43]. On the other hand, B cells also prevent neurodegeneration and axonal loss via producing brain-derived neurotrophic factor [44].
Intervention of common myeloid progenitor (CMP) cells' lineage in the immunopathogenesis of MS
T cells' secretion of chemokines, cytokines, MMPs, glutamate, and reactive oxygen species (ROS) facilitates their extravasation. This secretory response of T cells triggers an inflammatory cascade in the CNS, which then recruits other immune cells, including macrophages, B cells, and neutrophils. The active cascade persistently activates microglial and eventually results in demyelination, destruction of neurons axonal projections, and formation of sclerotic plaques in the white and gray matter [6, 26, 45-47].
DCs and MS
As professional APCs, DCs express high levels of MHC Ι/ΙΙ, as well as B7-1/CD80 and B7-2/CD86 co-stimulatory molecules [48]. In MS patients, DCs are profoundly resided in inflamed lesions and produce elevated levels of TNF-α, IFN-γ, IL-6, IL-12, and glutamate [26, 46] (Figure 1).
Neutrophils and MS
In MS patients, an increased number of circulating neutrophils is observed. The CXCR2, the main receptor of neutrophil-attracting chemokines CXCL1 and CXCL5, facilitates neutrophils migration and infiltration into the CNS. Likewise, the severity of MS disease attacks is tightly correlated to the overexpression of CXCL1/5 that justifies the role of neutrophils in CNS damage and the pathogenesis of MS [49]. Neutrophils undergo a process named NETosis. Through this process, neutrophils form web-like structures, known as neutrophils extracellular traps (NETs), composed of decondensed chromatin and a wide range of granular enzymes. The formation of NETs can be induced by infectious agents and immune complexes, cytokines, and chemokines (IL-8, TNF) [50]. Previous studies have been pointed to the significant roles of NETs in MS pathogenesis [51]. NETs contain factors such as myeloperoxidase (MPO) and neutrophil elastase that were increased in the plasma of patients with MS and correlated with MS lesion burden [49].
Imbalance of M1/M2 macrophages and microglial cells and their correlation to MS
Macrophages are divided into classically activated M1 or alternatively activated M2, depending on their pro-inflammatory or anti-inflammatory cytokine expression profile. These are two major phenotypes of macrophages that are frequently found in active and chronic MS plaques. The M1 macrophage reactivates peripheral and infiltrated T cells via antigen presentation. These cells also augment Th1 and Th17 responses via the secretion of pro-inflammatory cytokines IL-12, IL-23, IL-6, IL-1β, and TNF-α. Besides, M1 macrophages produce toxic mediators such as iNOS, NO, ROS, and glutamate and induce "oxidative stress," resulting in oligodendrocyte impairment, myelin degeneration, and ultimately neuronal loss [30, 46].
Microglial, as well as macrophages, are categorized into pro-inflammatory M1 and anti-inflammatory M2 phenotypes. Microglial are involved with phagocytosis of myelin debris and promoting the oligodendrocyte progenitor cells proliferation, which both are necessary for initiation of remyelination. Both of these processes are required for the alleviation of disease symptoms. Microglial also produces iNOS and activated radicals (NO, ROS), promoting oxidative damage of myelin sheath and neurons [52, 53]. Down-regulation of PU.1, as the master transcription regulator for myeloid cell lineage development, results in the suppression of macrophage and microglial differentiation [54, 55]. Recent studies show that interferon-regulatory factor 5/4 (IRF5/4) mediates activated M1/M2 phenotype polarization in macrophages and microglial. Indeed, the IRF5/4 regulatory axis plays a crucial role in balancing M1/M2 states in macrophages and microglial. Under inflammatory conditions, the aberrant expression of IRF5/4 leads to disturbance of M1/M2 balance [56-58] (Figure 1).
Biotherapeutic development in MS disease
Biotherapeutics approved by the Food and Drug Administration (FDA) or studied in clinical trials target RRMS (RMS) or PPMS patients. Therapies that target RMS focus on immunomodulation, while PPMS targeted therapies mainly deal with neurodegenerative components [59-63].
Of biotherapeutics that tested against RMS, Atacicep, Alemtuzumab, and Natalizumab targeted BAFF/APRIL (B cell activating factor/A proliferation-inducing ligand), CD52 (cluster of differentiation 52; glycoprotein antigen on the surface of mature lymphocyte), and integrins (transmembrane receptors that are involved in extracellular matrix adhesion), respectively, while Ofatumumab and Ocrelizumab targeted CD20 (B lymphocyte antigen CD20). The ocrelizumab is an FDA-approved biotherapeutic that is tested for PPMS as well. The contribution of B cells, which provides secretion of pro-inflammatory cytokines, facilitates the effective impact of ocrelizumab on both RMS and PPMS patients. All biotherapeutics that were tested against RMS or PPMS were different types of IgGs. The HLADRB1 locus and other genetic loci (e.g., IL-2 and CD25) are closely correlated to immunity's importance in MS [59]. Immunomodulation in RMS patients resulted in rather successful clinical benefits than PPMS. This finding points out the fact that "there might be immune contributions in PPMS patients that are neglected in targeted therapy of MS” [64]. Our survey shows that the imbalance between pathogenic and protective impacts of B cells in MS disease [30] is less complicated than what happens between M1/M2, Th2/Th1, and Treg/Th17 cells. It might explain why B cells that targeted therapies (via IgG mAbs) are more frequently used in the design of clinical trials. However, other therapies targeting either macrophage or T cell-secreted molecules suffer discordance between IL-12/23- and IL-17-targeted therapies [65]. To deal with this challenge, one can think of two strategies. First, focus on lymphocyte extravasation from the blood into the CNS. Second, T cell-targeted therapies directly target T cell-secreted interleukins. It seems that targeting T cells that express α4β1 in MS (first strategy) is rather promising than the second strategy, both as a matter of efficiency and further possible side effects. The Natalizumab is a successful example of such a case that works as an α4 blocking monoclonal antibody, approved for treating patients with RMS [66].
Conclusion
In autoimmune diseases like MS, the ability of the immune system to set a balance between pro-inflammatory and anti-inflammatory responses is lost. As progenitor cell lineages originating from a hematopoietic stem cell, both CLP and CMP descendants are involved in immune-pathogenesis of MS (Figure 1). CLP descendants develop into various T and B cells (e.g., Th1, Th2, Th17, Treg, NKT, γδ T, and MAIT), while CMP descendants develop neutrophils, dendritic cells, macrophages, and microglial cells. The CD8+ T cells (i.e., cytotoxic T cells) facilitate inflammation in CNS of RRMS patients, as well as γδ T and MAIT cells, while B cells are found in MS lesions of either RRMS or PPMS patients.
In other words, the imbalance between T cells (Th1/Th2, Th17/Treg T cells) comes up with secretion of chemokines, and cytokines, which is a trigger for the start of an inflammatory cascade in the CNS, which then recruits other immune cells including macrophages, B cells, and neutrophils. That is how macrophages and B cells, as downstream cells of the inflammatory cascade, can play both pathogenic and protective roles in MS disease.
As mentioned earlier, the imbalance between pathogenic and protective impacts of B cells in MS progression is less complicated than what happens between T cells and macrophages (M1/M2). It explains why most biotherapeutics that have been tested against RMS or PPMS patients in clinical trials are different types of IgG mAbs. The B cell-targeted therapies (via IgG mAbs) have been frequently used in clinical trials, which are more efficient than T-cell-targeted therapies while leaving the least possible side effects. However, targeting T cells in RMS/PPMS patients is more limited, and T cell lymphocyte extravasation from the blood into the CNS is known as the only promising strategy.
Conflicts of Interest
The authors declare that they have no conflict of interest.
Acknowledgments
We would like to thank the Stem Cell Technology Research Center (Tehran, Iran), especially the Department of Molecular Biology, for their support.
References
- Mansouri S, Zayeri F. Global and regional trends of multiple sclerosis disability-adjusted life years rates: a 25-year assessment. Neuroepidemiol. 2019; 52(1): 17-24.
- Lublin FD, Reingold SC, Cohen JA, Cutter GR, Sørensen PS, Thompson AJ, et al. Defining the clinical course of multiple sclerosis: the 2013 revisions. Neurol. 2014; 83: 278-86.
- McDonald WI, Compston A, Edan G, Goodkin D, Hartung HP, Lublin FD, et al. Recommended diagnostic criteria for multiple sclerosis: Guidelines from the international panel on the diagnosis of multiple sclerosis. Ann Neurol. 2001; 50(1): 121-27.
- Miller D, Barkhof F, Montalban X, Thompson A, Filippi M. Clinically isolated syndromes suggestive of multiple sclerosis, part I: natural history, pathogenesis, diagnosis, and prognosis. Lancet Neurol. 2005; 4(5): 281-88.
- Miller D, Barkhof F, Montalban X, Thompson A, Filippi M. Clinically isolated syndromes suggestive of multiple sclerosis, part 2: non-conventional MRI, recovery processes, and management. Lancet Neurol. 2005; 4(6): 341-48.
- Kaskow BJ, Baecher-Allan C. Effector T cells in multiple sclerosis. Cold Spring Harb Perspect Med. 2018; 8(4): 29025.
- Alinezhad-Bermi S, Kabiri M, Rad I, Irani S, Hanaee-Ahvaz H. Hyperosmolarity benefits cartilage regeneration by enhancing expression of chondrogenic markers and reducing inflammatory markers. In Vitro Cellular & Developmental Biology-Animal. 2021; 1(1): 1-10.
- Mittal K, Eremenko E, Berner O, Elyahu Y, Strominger I, Apelblat D, et al. CD4 T cells induce a subset of MHCII-expressing microglia that attenuates alzheimer pathology. Science. 2019; 16: 298-311.
- Volpe E, Sambucci M, Battistini L, Borsellino G. Fas-Fas ligand: checkpoint of T cell functions in multiple sclerosis. Front Immunol. 2016; 7(382): 1-9.
- Rocha NP, Colpo GD, Bravo-Alegria J, Lincoln JA, Wolinsky JS, Lindsey JW, et al. Exploring the relationship between endothelin-1 and peripheral inflammation in multiple sclerosis. J Neuroimmunol. 2019; 326(1): 45-8.
- Tavakolpour S, Tavakolpour V. Interleukin 4 inhibition as a potential therapeutic in pemphigus. Cytokine. 2015; 77(1): 189-195.
- Kalra S, Lowndes C, Durant L, Strange RC, Al-Araji A, Hawkins CP, et al. Th17 cells increase in RRMS as well as in SPMS, whereas various other phenotypes of Th17 increase in RRMS only. Multiple Sclerosis J Experiment, Translat Clin. 2020; 6(1): 1-10.
- Tahmasebinia F, Pourgholaminejad A. The role of Th17 cells in auto-inflammatory neurological disorders. Prog Neuropsycho-pharmacol Biol Psychiat. 2017; 79(3): 408-416.
- Setiadi AF, Abbas AR, Jeet S, Wong K, Bischof A, Peng I, et al. IL-17A is associated with the breakdown of the blood-brain barrier in relapsing-remitting multiple sclerosis. J Neuroimmunol. 2019; 332(7): 147-54.
- Wu B, Wan Y. Molecular control of pathogenic Th17 cells in autoimmune diseases. Int Immunopharmacol. 2020; 80: 106187.
- Hoesel B, Schmid JA. The complexity of NF-κB signaling in inflammation and cancer. Mol Cancer. 2013; 12(86): 1-5.
- Alavijeh MS, Maghsoudpour A, Khayat M, Rad I, Hatamie S. Cobalt ferrite decoration of molybdenum disulfide nanosheets; development of a nanocomposite-mediated hyperthermia method. J Mech Sci and Technol. 2021; 1(1): 1-7.
- Alavijeh MS, Bani MS, Rad I, Hatamie S, Zomorod MS, Haghpanahi M. Antibacterial properties of ferrimagnetic and super-paramagnetic nanoparticles: a comparative study. J Mech Sci Technol. 2021; 35(2): 815-21.
- Kurschus FC. T cell mediated pathogenesis in eae: molecular mechanisms. Biomed J. 2015; 38(3): 183-93.
- Kostić M. Role of Th1 and Th17 immune responces in pathogenesis of multiple sclerosis. Acta Medica Medianae. 2010; 49(4): 61-9.
- Soltanmoradi S, Tavakolpour V, Moghadasi AN, Kouhkan F. Expression analysis of NF-κB-associated long noncoding RNAs in peripheral blood mononuclear cells from relapsing-remitting multiple sclerosis patients. J Neuroimmunol. 2021; 356(7): 577602.
- Lee PW, Severin ME, Lovett-Racke AE. TGF-β regulation of encephalitogenic and regulatory T cells in multiple sclerosis. Eur J Immunol. 2017; 47(4): 446-53.
- Kuwabara T, Ishikawa F, Kondo M, Kakiuchi T. The role of IL-17 and related cytokines in inflammatory autoimmune diseases. Mediators Inflamm. 2017; 20(2): 1-11.
- Zhu L, Hua F, Ding W, Ding K, Zhang Y, Xu C. The correlation between the Th17/Treg cell balance and bone health. Immunity Ageing. 2020; 17(30): 1-10.
- Vasileiadis GK, Dardiotis E, Mavropoulos A, Tsouris Z, Tsimourtou V, Bogdanos DP, et al. Regulatory B and T lymphocytes in multiple sclerosis: friends or foes?. Autoimmunity Highlights. 2018; 9(1): 1-5.
- Dargahi N, Katsara M, Tselios T, Androutsou ME, de Courten M, Matsoukas J, et al. Multiple sclerosis: immunopathology and treatment update. Brain Sci. 2017; 78(7): 1-27.
- Danikowski KM, Jayaraman S, Prabhakar BS. Regulatory T cells in multiple sclerosis and myasthenia gravis. J Neuroinflam. 2017; 14(117): 1-16.
- Luo C, Jian C, Liao Y, Huang Q, Wu Y, Liu X, et al. The role of microglia in multiple sclerosis. Neuropsychiatric disease and treatment. 2017; 13: 1661-667.
- van Langelaar J, van der Vuurst de Vries RM, Janssen M, Wierenga-Wolf AF, Spilt IM, Siepman TA, et al. T helper 17.1 cells associate with multiple sclerosis disease activity: perspectives for early intervention. Brain: J Neurol. 2018; 141(5): 1334-349.
- Yong H, Chartier G, Quandt J. Modulating inflammation and neuroprotection in multiple sclerosis. J Neuro Res. 2017; 96(6): 927-50.
- Berge T, Eriksson A, Brorson IS, Høgestøl EA, Berg‑Hansen P, Døskeland A, et al. Quantitative proteomic analyses of CD4+ and CD8+ T cells reveal differentially expressed proteins in multiple sclerosis patients and healthy controls. Clin Proteom. 2019; 16(19): 1-18.
- Jones AP, Kermode AG, Lucas RM, Carroll WM, Nolan D, Hart PH. Circulating immune cells in multiple sclerosis. Clin Exp Immunol. 2016; 187(2): 193-203.
- Kaer LV, Wu L, Parekh VV. Natural killer T cells in multiple sclerosis and its animal model, experimental autoimmune encephalomyelitis. Immunol. 2015; 146(1): 1-10.
- Cui Y, Wan Q. NKT cells in neurological diseases. Front Cel Neurosci. 2019; 13(245): 1-10.
- Latha TS, Reddy MC, Durbaka PVR, Rachamallu A, Pallu R, Lomada D. γδ T cell mediated immune responses in disease and therapy. Front Immunol. 2014; 5(571): 1-11.
- Malik S, Want MY, Awasthi A. The emerging roles of gamma–delta T cells in tissue inflammation in experimental autoimmune encephalomyelitis. Front Immunol. 2016; 7(14): 1-11.
- Spahn TW, Issazadah S, .Salvin AJ, Weiner HL. Decreased severity of myelin oligodendrocyte glycoprotein peptide 33 – 35-induced experimental autoimmune encephalomyelitis in mice with a disrupted TCR δ chain gene. Euro J Immunol. 1999; 29(12): 4060-4071.
- Monteiro A, Cruto C, Rosado P, Martinho A, Rosado L, Fonseca M, et al. Characterization of circulating gamma-delta T cells in relapsing vs remission multiple sclerosis. J Neuroimmunol. 2018; 318(1): 65-71.
- Contentti EC, Farez MF, Correale J. Mucosal-associated invariant T cell features and TCR repertoire characteristics during the course of multiple sclerosis. Front Immunol. 2019; 10(2690): 1-17.
- Hinks TSC, Zhang XW. MAIT cell activation and functions. Front Immunol. 2020; 11(1014): 1-10.
- Zhou Y, Zhang Y, Han J, Yang M, Zhu J, Jin T. Transitional B cells involved in autoimmunity and their impact on neuroimmunological diseases. J Translat Med. 2020; 18(1): 1-12.
- Bittner S, Ruck T, Wiendl H, Grauer OM, Meuth SG. Targeting B cells in relapsing–remitting multiple sclerosis: from pathophysiology to optimal clinical management. Ther Adv Neurol Disord. 2017; 10(1): 51-66.
- Gharibi T, Babaloo Z, Hosseini A, Marofi F, Ebrahimi-Kalan A, Jahandideh S, et al. The role of B cells in the immunopathogenesis of multiple sclerosis. Immunology. 2020; 160(4): 325-35.
- Xiao J. Neuroprotection on multiple sclerosis: A BDNF perspective. J Neurol Neurophysiol. 2012; 3(3): 108.
- Solleiro-Villavicencio H, Rivas-Arancibia S. Effect of chronic oxidative stress on neuroinflammatory response mediated by CD4+T cells in neurodegenerative diseases. Front Cell Neurosci. 2018; 12(114): 1-13.
- Levite M. Glutamate, T cells and multiple sclerosis. J Neural Transm. 2017; 124(7): 775-98.
- Khodayari K, Alipour M, Rad I, Ramshini H, Abdolmaleki P. Inhibition potential evaluation of two synthetic bis-indole compounds on amyloid fibrillation: a molecular simulation study. J Biomol Struct Dyn. 2020; 2(12): 1-11.
- Tai Y, Wang Q, Korner H, Zhang L, Wei W. Molecular mechanisms of T cells activation by dendritic cells in autoimmune diseases. Front Pharmacol. 2018; 9(642): 1-10.
- Rumble JM, Huber AK, Krishnamoorthy G, Srinivasan A, Giles DA, Zhang X, et al. Neutrophil-related factors as biomarkers in EAE and MS. J Experiment Med. 2015; 212(1): 23-35.
- Vorobjeva NV, Chernyak BV. NETosis: molecular mechanisms, role in physiology and pathology. Biochem. 2020; 85(10): 1178-1190.
- Fousert E, Toes R, Desai J. Neutrophil extracellular traps (NETs) take the central stage in driving autoimmune responses. Cells. 2020; 9(4): 915.
- Chu F, Shi M, Zheng C, Shen D, Zhu J, Zheng X, et al. The roles of macrophages and microglia in multiple sclerosis and experimental autoimmune encephalomyelitis. J Neuroimmunol. 2018; 15(318): 1-7.
- Voet S, Prinz M, Loo Gv. Microglia in central nervous system inflammation and multiple sclerosis pathology. Trends Mol Med. 2019; 25(2): 112-123.
- Guerrero BL, Sicotte NL. Microglia in multiple sclerosis: friend or foe?. Front. Immunol. 2020; 11(374): 1-8.
- Crotti A, Ransohoff RM. Microglial physiology and pathophysiology: Insights from genome-wide transcriptional profiling. Immunity. 2016; 44(3): 505-515.
- Chistiakov DA, Myasoedova VA, Revin VV, Orekhov AN, Bobryshev YV. The impact of interferon-regulatory factors to macrophage differentiation and polarization into M1 and M2. Immunobiol. 2018; 223(1): 101-111.
- Mamun AA, Chauhan A, Yu H, Xu Y, Sharmeen R, Liu F. Interferon regulatory factor 4/5 signaling impacts on microglial activation after ischemic stroke in mice. Eur J Neurosci. 2018; 47(2): 140-49.
- Rad I, Honardoost M. Structural modification in hepatitis c virus envelope protein; potential viral strategy against interferon therapy. International J Peptide Res Therapeut. 2020; 26(1): 171-79.
- Ghilardi N, Pappu R, Arron JR, Chan AC. 30 Years of biotherapeutics development-what have we learned?. Ann Rev Immunol. 2020; 38(3): 249-87.
- Torabi S, Tamaddon M, Asadolahi M, Shokri G, Tavakoli R, Tasharrofi N, et al. miR-455-5p downregulation promotes inflammation pathways in the relapse phase of relapsing-remitting multiple sclerosis disease. Immunogenetics. 2019; 71(2): 87-95.
- Tavakolpour V, Shokri G, Moghadasi AN, Nahavandi PM, Hashemi M, Kouhkan F. Increased expression of mir-301a in PBMCs of patients with relapsing-remitting multiple sclerosis is associated with reduced NKRF and PIAS3 expression levels and disease activity. J Neuroimmunol. 2018; 325(12): 79-86.
- Karami S, Kouhkan F, Rad I, Tavakolpoor Saleh N, Shokri G, Fallah P, et al. The miR-561 suppresses glioblastoma cell proliferation through C-myc regulation. Mid East J Cancer. 2021; 12(3): 321-31.
- Mona T, Gelareh S, Ali HRSM, Iman R, Àmirnader ER, Fatemeh K. Involved microRNAs in alternative polyadenylation intervene in breast cancer via regulation of cleavage factor “CFIm25”. Scientific Rep. 2020; 10(1): 11608.
- Montalban X, Hauser SL, Kappos L, Arnold DL, Bar-Or A, Comi G, et al. Ocrelizumab versus placebo in primary progressive multiple sclerosis. New Eng J Med. 2017; 376(3): 209-220.
- Minton K. IL-17A brings new recruits to EAE. Nat Rev Immunol. 2020; 20(3): 137-45.
- Chataway J, De Angelis F, Connick P, Parker RA, Plantone D, Doshi A, et al. Efficacy of three neuroprotective drugs in secondary progressive multiple sclerosis (MS-SMART): a phase 2b, multiarm, double-blind, randomised placebo-controlled trial. Lancet Neurol. 2020; 19(3): 214-25.